Green Synthesis of Iron Oxide Nanoparticles from Coriandrum sativum L. Extract and it's Potential Use in MRI and Medical Imaging.
- ACS BCP
- Feb 11, 2024
- 6 min read
INTRODUCTION:
Iron oxide nanoparticles (IONPs) have emerged as versatile contrast agents for magnetic resonance imaging (MRI) owing to their unique magnetism and biocompatibility. However, traditional contrast agents exhibit fixed enhanced signals, limiting sensitivity and specificity. Stimulus-responsive IONPs offer a solution by altering relaxation signals in response to internal or external stimuli, overcoming these limitations. While IONPs find applications in various fields such as catalysis and pollutant degradation, conventional synthesis methods often yield non-environmentally friendly and expensive nanoparticles prone to agglomeration. Phyto-mediated synthesis, using plant extracts as reducing and capping agents, offers a clean, fast, and eco-friendly alternative. Coriandrum sativum L. leaf extract has been utilized for rapid and stable synthesis of iron oxide nanoparticles (FeONPs), demonstrating the potential of environmentally benign methods in nanoparticle production. For this article, the acronyms IONPs, FeONPs and SPIONs are used interchangeably as they are synonymous to each other.
PREPARATION OF IONPs FROM C. SATIVUM EXTRACT:
The synthesis of iron oxide nanoparticles (FeONPs) through the utilization of Coriandrum sativum L. leaf extract represents a novel and environmentally friendly approach. Initially, fresh C. Sativa L. leaves were meticulously collected and washed with both tap water and deionized water to ensure purity. Subsequently, a meticulously prepared aqueous leaf extract was obtained by combining 10 g of the plant leaf, cut into small pieces, with 100 mL of deionized water in a 250 mL beaker, followed by heating the solution to 60°C for 1 hour. The resultant solution was then cooled and meticulously filtered using Whatman No. 1 filter paper to ensure clarity. Further purification was achieved through centrifugation, followed by freeze-drying to obtain a powdered form of the extract, which was sieved and stored under appropriate conditions for further use. The biosynthesis of FeONPs ensued by the gradual addition of C. sativum leaf extract to a solution containing 0.01 (mM) FeSO4 in a conical flask. This step was accompanied by constant agitation at 1000 rpm for 15 minutes at 30°C, facilitated by a magnetic stirrer. Post-synthesis, the FeONPs were separated via centrifugation at 15000 rpm for 20 minutes, and the resulting pellet underwent thorough purification through multiple washes with triple distilled water. Subsequently, the purified FeONPs were subjected to freeze-drying for 24 hours at -78°C under a pressure of 10 Pa to obtain a stable and dry form suitable for further analysis and application.
ANALYSIS OF THE SPIONS FORMED
Characterization of the synthesized FeONPs involved comprehensive testing and evaluation criteria. Transmission electron microscopy (TEM) analysis revealed the nearly spherical and monodisperse nature of the nanoparticles, with minimal agglomeration, indicating successful formation and stabilization. This underscores the efficacy of phyto-constituents present in C. sativum L. leaves, acting as efficient reducing and capping agents. Additionally, magnetic properties were assessed using a vibrating sample magnetometer (VSM) at room temperature within a magnetic field ranging from -16,000 to 16,000 Oe. The measured saturation magnetization (Ms), remnant magnetization (Mr), and coercivity (Ce) values provided insights into the superparamagnetic nature of the green-synthesized FeONPs, along with the absence of long-range magnetic dipole-dipole interactions among the assemblies of superparamagnetic nanoparticles. Moreover, the FeONPs demonstrated superparamagnetic behaviour, as evidenced by the absence of a hysteresis loop in the magnetometry measurements. The saturation magnetization (Ms) of the FeONPs was measured to be 3.483 emu/g, while the remnant magnetization (Mr) and coercivity (Ce) values were 45.389 emu/g and 62.969G, respectively.
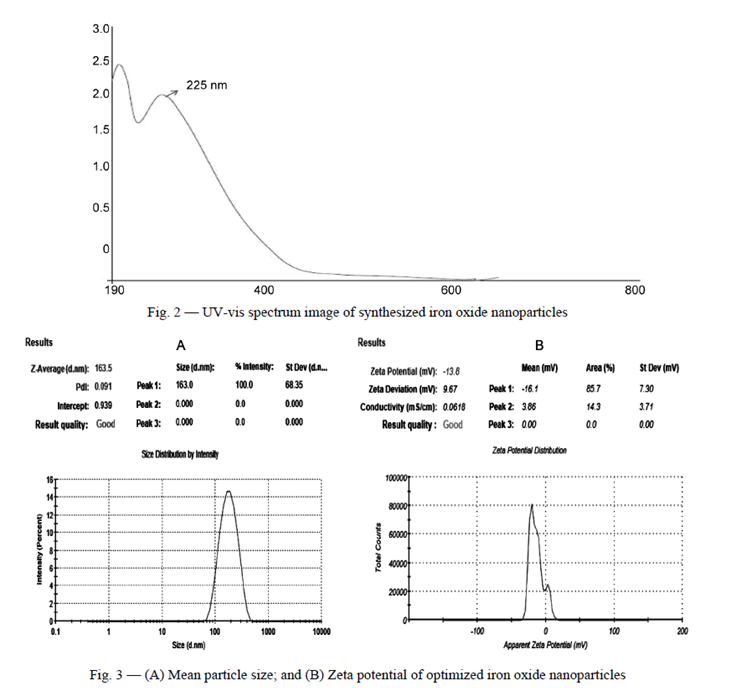
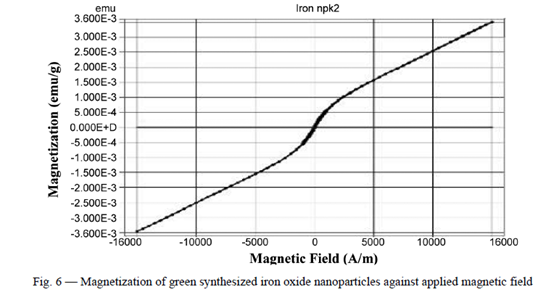
APPLICATIONS OF SPIONS IN MRI AND MEDICAL IMAGING:
MRI measures the time for the magnetic moments of primarily hydrogen protons to return to their equilibrium state, a slight net alignment parallel to a strong external magnetic field (z-direction), after being rotated 90 degrees into the transverse (x-y) plane by an orthogonal radiofrequency (RF) pulse. This recovery process is characterized by two relaxation times: the spin-lattice, or longitudinal relaxation (designated as T1) and the spin-spin or transverse relaxation (designated as T2). T1 relaxation occurs when spins lose energy by interacting with their environment (hence, spin-lattice), thus increasingly favouring an energetically preferable alignment with the external magnetic field. A contrast agent facilitates this energy loss through dipole-dipole interactions between water molecules and metal ions in the contrast agent’s core. Energy exchange happens most efficiently at the Larmor frequency (42.58 MHz/T for hydrogen protons), therefore T1 contrast agents are most effective when their rotational motion matches this frequency. The rapid tumbling time of water molecules is slowed to nearer the Larmor frequency by interaction with the contrast agent, allowing hydrogen protons to lose energy and recover their initial magnetic moment faster. T2 relaxation reflects a loss of the net magnetization in the transverse plane, where the initial net alignment with the external magnetic field becomes a net phase coherence of their precession in the transverse plane, and thus a small magnetic moment. All processes causing T1 relaxation will also cause the precession of these spins to decohere, but they are not the only mechanisms affecting T2 relaxation. Contrast agents may impact T2 by creating local magnetic field inhomogeneities. The induced fields create regions where the Larmor frequency, which depends on field strength, differs from that of the applied longitudinal field. As this characteristic precession of hydrogen protons is altered, the spins fall out of phase, reducing any net transverse magnetization. The relaxation rates, denoted as r1 and r2 relaxivities, are the inverse of the T1 and T2 relaxation times, respectively. It is important to note that the ratio of r2/r1 is a key parameter in distinguishing T2 and T1 contrast agents. T2 contrast agents possess high r2 relaxivity, hence have a large r2/r1 ratio. In comparison, T1 contrast agents should have high r1 relaxivity, with the r2/r1 ratio being smaller, ideally close to 1.[9] Therefore, creating a T1 contrast agent involves minimizing the r2/r1 ratio, which can be achieved by decreasing r2 and increasing r1.
pH-triggered disassembly behaviour can change the size of IONP clusters, which significantly influences their imaging signal. This strategy has been to describe an efficient method to enhance contrast in tumours by delivering assembled IONPs into tumour sites by the enhanced permeability and retention (EPR) effect of larger particles, and then decomposing them under the acidic tumour microenvironment to recover the T1 properties of USPIO NPs. The assembled IONPs can be absorbed by normal tissue and present a strong T2 effect, and the disassembled particles in the tumour site have a strong T1 effect, which improves the contrast between the tumour site and the surrounding tissue. The IONP nanoclusters disassemble upon encountering the acidic tumour environment, demonstrating a significant drop in the r2 relaxivity and r2/r1 value when the pH is acidic. This strategy greatly enhanced the T1 MRI contrast between the normal liver and hepatocellular carcinoma (HCC) in vivo, providing a highly sensitive diagnostic method for small hepatocellular carcinoma. The studies reported another pH-sensitive IONP Nano system cross-linked by small-molecule ligands. At pH 7.4, IONAs are structurally robust, while under acidic conditions in tumour sites, IONAs were quickly decomposed into a large amount of hydrophilic USPIO NPs, producing a strong T1-weighted MR signal. At a low pH value of 5.5, r1 increased, and r2 decreased significantly. In addition, there have been reports of other disassembling methods that regulate the signals of IONPs, including encapsulating IONPs in hydrogels or polymeric micelles, which can decompose in the acidic conditions of the tumour, and then release IONPs to enhance the T1 contrast images of the tumour. These strategies provide a promising way to improve detection sensitivity through various ingenious designs.
However, the assembled IONPs were easily sequestered in the liver by Kupffer cells due to their larger size, posing a safety risk. Therefore, the long-term metabolic effects of RIAs in the liver should be explored before further application.
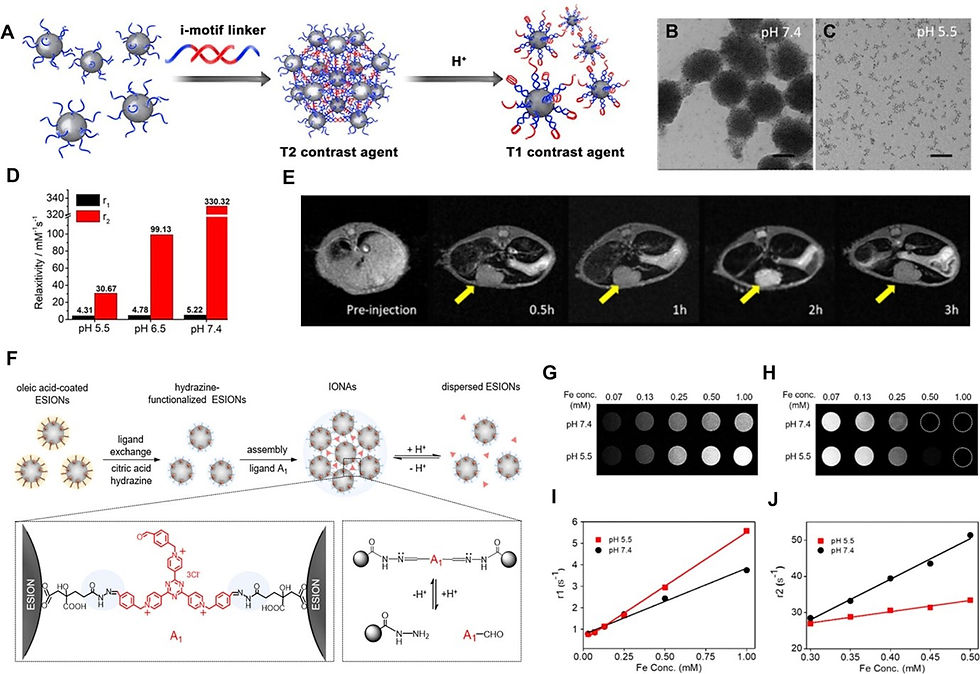
CONCLUSION:
In conclusion, the synthesis of iron oxide nanoparticles (FeONPs) utilizing Coriandrum sativum L. leaf extract presents a sustainable and environmentally friendly method. The meticulous preparation of the aqueous leaf extract ensured the purity and efficacy of the reducing and capping agents present in the plant material. The biosynthesis of FeONPs involved a gradual addition process, followed by purification and freeze-drying to obtain stable nanoparticles suitable for further analysis. Characterization of the synthesized FeONPs revealed their nearly spherical and monodisperse nature, along with superparamagnetic behaviour and absence of long-range magnetic interactions, as evidenced by transmission electron microscopy (TEM) and vibrating sample magnetometry (VSM). These findings underscore the potential of C. sativum L. leaf extract as an efficient agent for the synthesis and stabilization of FeONPs. Moreover, the application of FeONPs in pH-responsive MRI contrast enhancement for cancer imaging holds significant promise. pH-triggered disassembly behaviour of FeONPs enables efficient contrast enhancement in tumours by exploiting the acidic tumour microenvironment. This strategy has shown considerable potential in improving detection sensitivity and providing a highly sensitive diagnostic method for various cancers. However, challenges such as potential sequestration in the liver and long-term metabolic effects of FeONPs warrant further investigation and optimization before clinical application. Overall, the synergistic combination of green synthesis methods and pH-responsive contrast enhancement strategies represents a promising avenue for the development of advanced MRI contrast agents with enhanced diagnostic capabilities and reduced environmental impact.
REFERENCES:
1. Iron oxide nanoparticles as T1 contrast agents for magnetic resonance imaging: fundamentals challenges, applications, and prospective
Authors: Mike Jeon, Mackenzie V. Halbert, Zachary R. Stephen, and Miqin Zhang
2. Green synthesis and characterization of iron oxide nanoparticles using Coriandrum sativum L. leaf extract
Authors: Kuldeep Singh, Dimple Sethi Chopra*, Dhandeep Singh & Nirmal Singh
3. Designing Smart Iron Oxide Nanoparticles for MR Imaging of Tumours
Authors: Zhenzhen Li, Ru Bai, Jia Yi, Huige Zhou*, Junfang Xian*, and Chunying Chen*
Comments